We study proximity effects in complex oxides at the nanoscale. Oxides exhibit highly unique properties including collective phenomena such as magnetism, ferroelectricity, superconductivity, metal-insulator transitions, enhanced photoconductivity, and electron transfer. Oxides also play a key role in both energy and information technologies. Examples include batteries, solar cells, catalysis, and miscellaneous electronic and magnetic devices.
Our interest lies in the electronic, magnetic, optical, and structural properties of materials and devices. We are interested in studying how different properties of systems change on mesoscopic or nano-length scales. In addition, proximity effects and external stimuli are tools that allow us to tailor the properties of these systems.
The experimental tools which we apply are a combination of magnetic and transport measurements, structural characterization, and local probe measurements in various conditions. These conditions include applied temperature, magnetic field, gate voltages, stress, currents, and microwave excitations. In addition, we collaborate with various groups specialized in different techniques, such as synchrotron and neutron experiments, ultra-fast measurements, and Raman spectroscopy.
1. Tuning Quantum properties with size in electron-correlated oxides
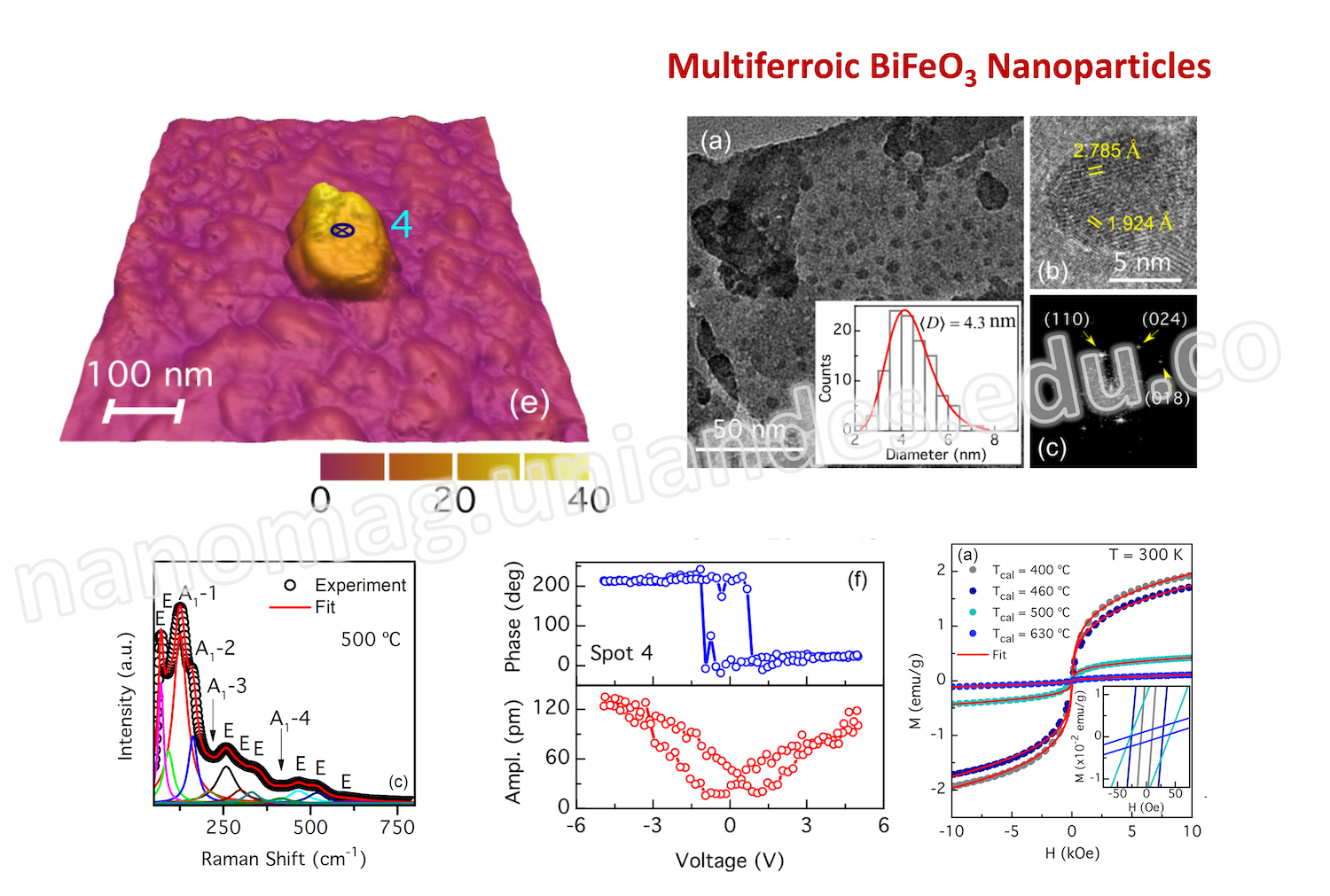
Electron-correlated oxides exhibit a plethora of multifunctional properties that have its root in the complex energy landscape due to several degrees of freedom. All these interactions have a characteristic length scale and therefore, one can enhance or diminish them controlling the sample dimensionality. We are interested in the resulting effects of confinement at the nanoscale, typically nanoparticles. As an example, we have proved that in ferroelectric BiFeO3, an antiferromagnetic material when in bulk, becomes ferromagnetic-like at sizes below 60 nm approx. Interestingly, the ferroelectric properties survived, and therefore, NPs of BFO is ferroelectric and ferromagnetic (multiferroic) at room temperature. We are exploring such properties for applications to neurotrophic and quantum computing.
[1.1] Carranza-Celis et. al., Scientific Reports 9, 3182 (2019).
[1.2] E. Ramos et. al., Strain-controlled ferromagnetism in BiFeO3 Nanoparticles, Journal of Physics: Condensed Matter. https://doi.org/10.1088/1361-648X/ab6b8a
2. Neuromorphic Computing based on Resistive Switching phenomena in Transition-metal Oxide systems
We are interested in novel approaches (devices and properties) that use the multifunctional properties of oxide materials. For instance, Resistive Switching is at the core of implementations of Neuromorphic computing and Oxide systems based on Transition Metal elements. We are studying materials with multifunctional properties as candidates for future implementations of neuromorphic architectures.
More information about the Resistive switching phenomena is here:
[2.1] J. del Valle, Juan Gabriel Ramírez, M. Rosenberg, Ivan K. Schuller, Challenges in materials and devices for Resistive-Switching-based Neuromorphic Computing, J. Appl. Phys. 124, 211101 (2018)
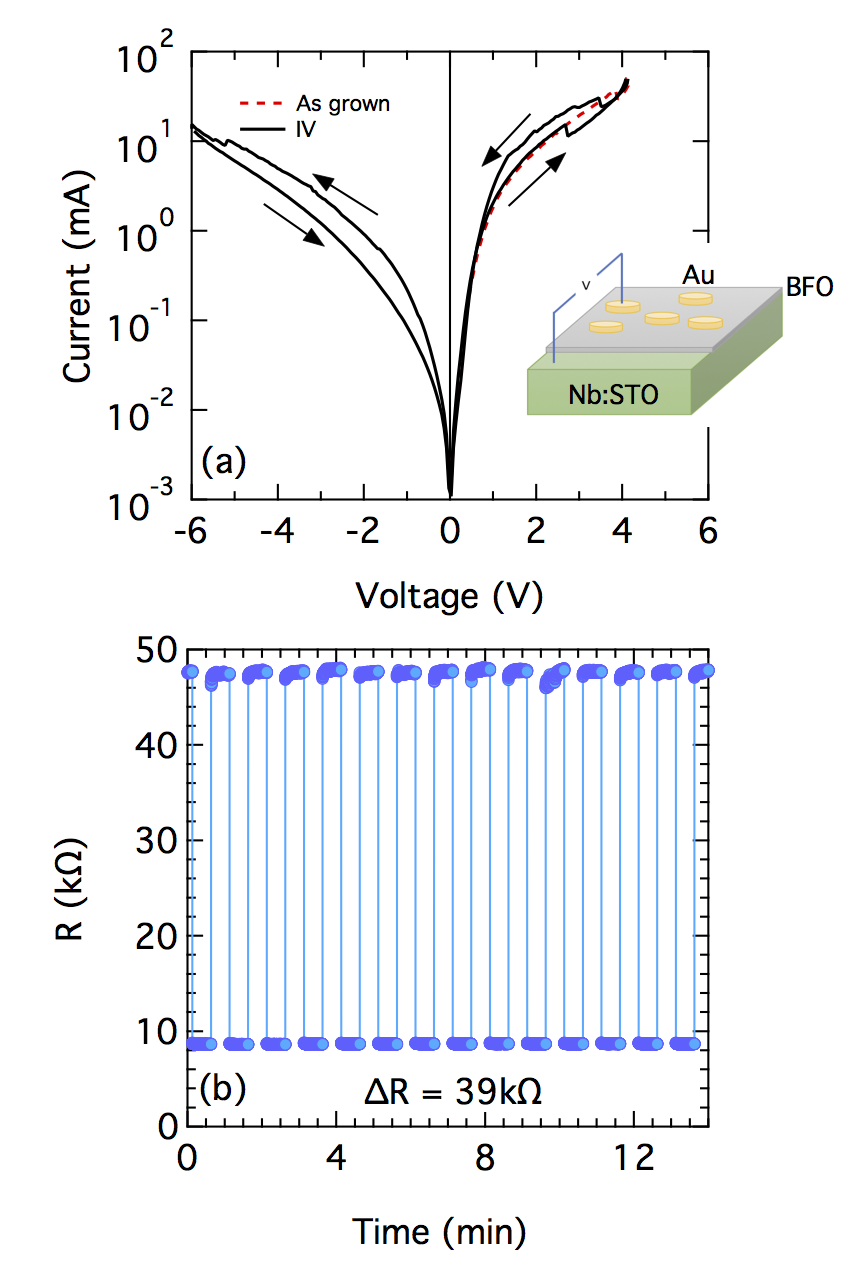
We have also shown that Ferroelectric BiFeO3 thin films present Resisting Switching phenomena that depend on the electric polarization and oxygen vacancy content.
[2.2] Alexander Cardona, Isabel C. Arango, Maria F. Gomez, Claribel Dominguez, Juan Trastoy, Christian Urban, Soumitra Sulekar, Juan C. Nino, Ivan K. Schuller, Maria E. Gomez, and Juan Gabriel Ramírez, Resistive Switching in Multiferroic BiFeO3 Films: Ferroelectricity vs vacancy migration. Solid State Communications 288 (2019) 38–42
More systems are being explored in our laboratory.
3. Control of phase transitions by disorder
A consequence of a first order phase transition is the phase coexistence across the order parameter. [1-3] This coexistence imposes onto the system a critical length scale resulting in critical phenomena, like avalanches. [1-3] We are interested in the role that defects or disorder plays in these systems when they are induced at length scales comparable to the phase coexistence. We have proven that materials undergoing Metal-Insulator transitions (MITs) respond to defects in a way that reveals their internal correlations. For example, We found that VO2 is more robust to the disorder that V2O3. [4] The disorder was induced by oxygen irradiation at low enough energies to prevent chemical doping. This was done in collaboration with Dr. Javier Villegas at CNRS. The robustness of the VO2 is a consequence of the V-V hybridization that occurs at the transition. Furthermore, we found that V2O3 has a disorder threshold below which the MIT is preserved. The existence of a threshold points to a global mechanism responsible for the MIT, as opposed to VO2, where local effects are crucial. All these effects emphasize the importance of disorder in electron-correlated systems. Open questions remain regarding the nano-scale effects of the disorder when induced in nano-structured systems [5]. These results suggest similar effects in a plethora of electron-correlated materials. Therefore We propose to look for disorder effects in other complex oxides with first-order phase transitions. Examples of these materials are Manganites [6], nickelates [7] and other transition metal oxides. Looking at the response to a controlled disorder of these complex oxides will shed light on the trigger mechanisms of their phase transitions. See reference [8] for more details.
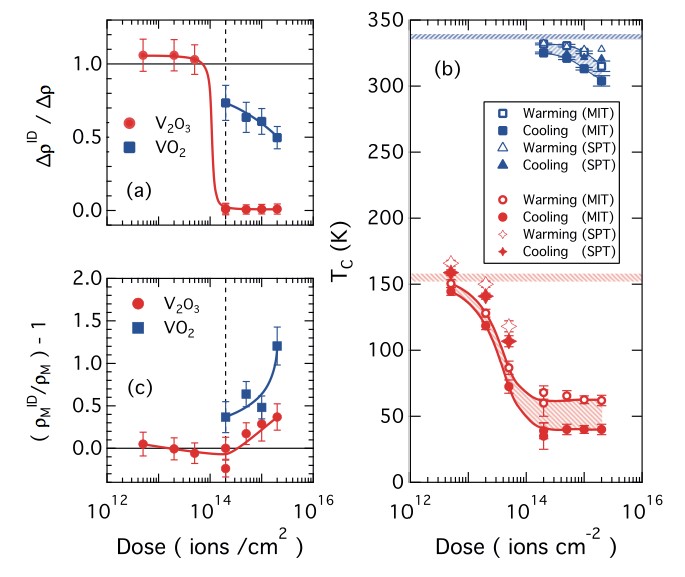
Complex oxides are often fabricated in dedicated sputtering and pulsed laser deposition systems. The former one has the ability to grow multiple materials, the last one, has the ability to be able to produce stoichiometric films. This means that the crystal symmetry is preserved from the target material. This has an enormous advantage when dealing with multi-element materials. Therefore, we expect to be able to purchase a PLD system with mix resources from Colciencias, FAPA, and grants from Uniandes.
[3.1] J. G. Ramirez, R. Schmidt, A. Sharoni, M. E. Gómez, I. K. Schuller, and E. J. Patiño, Appl. Phys. Lett. 102 (2013).
[3.2] J. G. Ramirez, A. Sharoni, Y. Dubi, M. E. Gómez, and I. K. Schuller, Physical Review B 79, 235110 (2009).
[3.3] A. Sharoni, J. Ramírez, and I. Schuller, in Phys. Rev. Lett. 101 026404 (2008).
[3.4] T. Saerbeck, G. Ramírez, S. Wang, and I. K. Schuller, in preparation (2015).
[3.5] V. Dobrosavljevic and G. Kotliar, Acta Physica Polonica A 85, 21 (1994).
[3.6] E. Dagotto, Science 309, 257 (2005).
[3.7] M. L. Medarde, J. Phys.: Condens. Matter 9, 1679 (1999).
[3.8] Juan Gabriel Ramirez, Thomas Saerbeck, Siming Wang, J. Trastoy, M. Malnou, J. Lesueur, Jean-Paul Crocombette, Javier E. Villegas, and Ivan K. Schuller, Physical Review B 91, 205123 (2015).
4. Magnonic control in hybrid materials
We demonstrated that the first-order phase transitions of complex oxides could be used to control the magnetization of ferromagnetic layers when in proximity. [9] Furthermore, we have found that the effects can be enhanced when the length scales of the magnetic and structural correlations are of the same order. [10] I have applied this idea to control collective magnetic excitations, which manifest as spin waves (or magnons), by enhancing their magnetic damping. [11] Measurements were done at microwave frequencies in a typical electron paramagnetic setup (EPR). We found that the phase coexistence in the oxide layer creates pinning centers that couple ferromagnetic modes to spin waves causing an increase in the spin-lattice relaxation time. This effect allows us to selectively control the magnetization dynamics by engineering materials with a variable phase-separation length scale. We propose to extend these studies to nanostructures materials in a wide range of frequencies (1 to 100 GHz), by employing coplanar waveguides. This will allow for devices with damping control. The use of coplanar waveguides will allow for a continuous broad-range variation of frequencies.
Currently, my laboratory has an available vector network analyzer (VNA), a microwave sweeper from 1 to 20 GHz, and a test parameter set. These instruments would allow studying the dynamical response of the magnetism in hybrid structures in a broader range of frequencies than the one available in an EPR setup. Additional components and equipment are in the process to be purchased to scale down to nano and micron scales.
[4.9] J. de la Venta, S. Wang, T. Saerbeck, J. G. Ramirez, I. Valmianski, and I. K. Schuller, Appl. Phys. Lett. 104, 062410 (2014).
[4.10] J. de la Venta, S. Wang, J. G. Ramirez, and I. K. Schuller, Appl. Phys. Lett. 102, 122404 (2013).
[4.11] Juan Gabriel Ramírez, J. de la Venta, Siming Wang, Thomas Saerbeck, Ali C. Basaran, X. Batlle, and Ivan K. Schuller, Phys. Rev. B 93, 214113 (2016).
4. Magnetism dynamics in complex oxides
We have demonstrated recently that magnetic percolation controls the magnetism dynamics in LPCMO thin films. This extraordinary behavior can be attributed to phase coexistence.
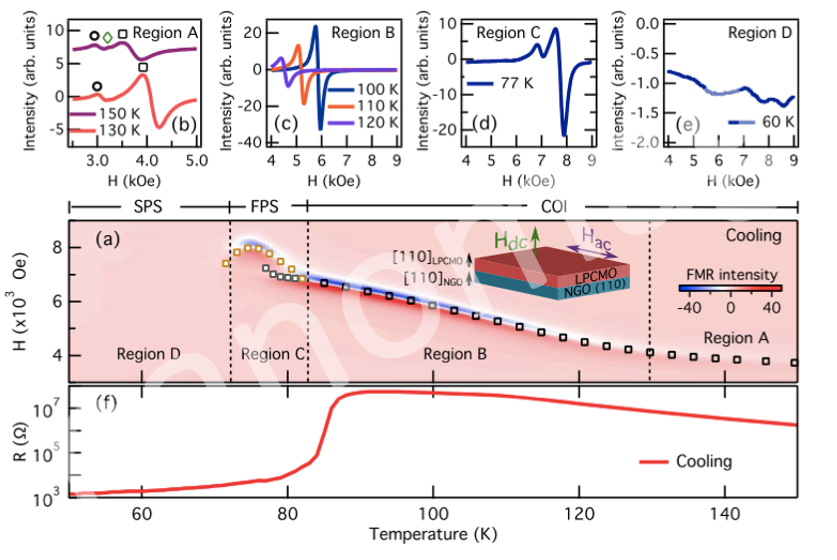